HPF has validated its first planet called G 9-40b, a sub-Neptune sized planet (2 Earth radii) orbiting a nearby low mass M-dwarf star with an orbital period of 6 days. The paper has been published in the Astronomical Journal and is available freely on the arXiv preprint server here.
It takes a Village to Validate a Planet
What does it take to say that a planet candidate is a bone-fide planet? Even for promising planet candidates around nearby stars, it takes a number of follow-up observations to say that a planet candidate is really a planet, and not something else, such as an eclipsing binary star system or other astrophysical false positives that can imitate planet transits.
G 9-40 was originally presented to have a candidate transiting planet in a paper by Yu et al. 2018 using photometric data from the K2 mission. After a closer look at this candidate planet, we saw it looked interesting for many reasons.
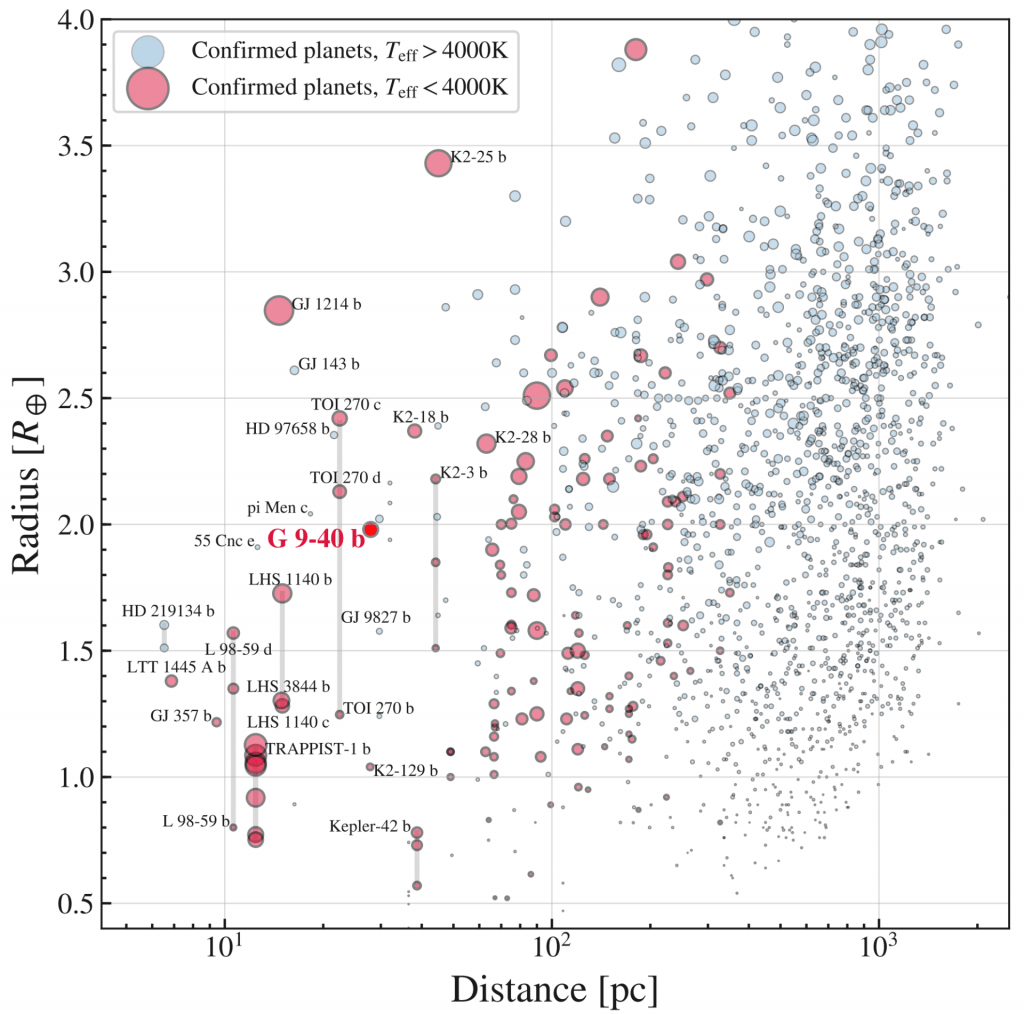
Figure 1: Planet radius as a function of the distance of the planet system from Earth in parsecs (1 parsec is about 3.2 light years). G 9-40b (highlighted in the bright red) is among the closest transiting systems known to date. Red points show planets around low mass stars (M-dwarfs) and blue points show planets orbiting more massive hotter stars. Figure 12 from the paper.
Given these reasons, we decided to conduct more observations of this planet candidate and characterize it better to decide if this was a planet or not. To do so we used Adaptive Optics (AO) imaging observations, further precision transit follow-up observations from the ground, and precision radial velocities with HPF. Lets dive into each of these observations below.
Adaptive Optics Imaging
To check if G 9-40 is blended with another star—which could confuse us on which star is the true source of the transit, or indicate that the system would be an eclipsing binary system rather than a true planet—we used high contrast adaptive optics imaging observations from the ShaneAO system at Lick Observatory. These adaptive optics observations are often conducted by shooting a high power laser into the air (Figure 3) to generate a so-called laser guide star. This laser guide star is then used to correct for the distortion in the atmosphere by rapidly changing the shape of an ‘adaptive’ mirror in the optical system, allowing us to obtain extremely high contrast images of the star. From these observations we see no evidence of blending or other stellar companions nearby the star G 9-40, further suggesting that G 9-40 is the true source of the transits we see from K2 and in our ground-based data (see next section).
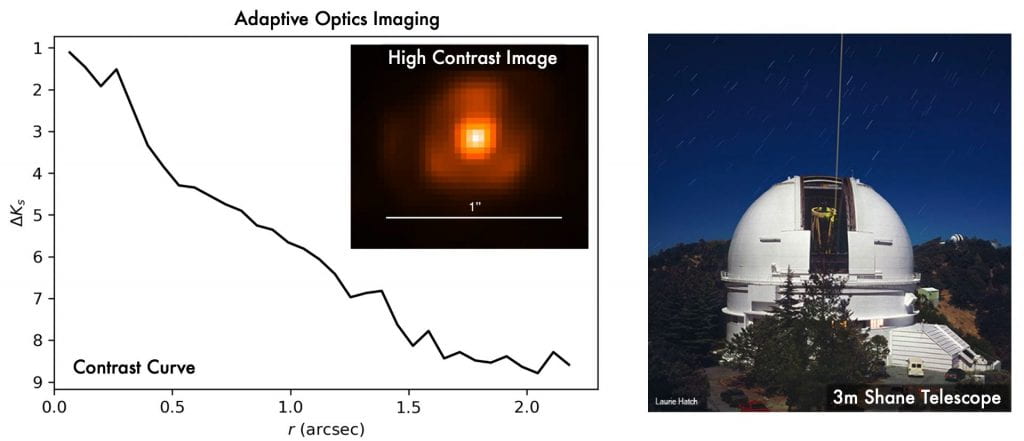
Figure 3: Adaptive optics image (left inset) and resulting contrast curve as observed with the ShaneAO Adaptive Optics (AO) System on the 3m Shane Telescope at Lick Observatory (right). The contrast curve on the left shows the brightness of the inset image (in the near-infrared K-band) as a function of the distance from the center of the star. We see no evidence of a background star in the image that could dilute and/or be the source of the transits we see in the K2 and/or ground-based data. Left: Figure 1 from the paper. Right image credit: Laurie Hatch.
Ground-based Transit Photometry using Engineered Diffusers
To constrain the planet parameters and further update the orbital ephemeris of the system, we used high-precision diffuser-assisted photometry using the ARCTIC imager on the 3.5m Telescope at Apache Point Observatory. We observed the transit using a custom-built narrow-band filter made by Semrock / AVR Optics that operates in a band with little-to-no water absorption. We often call this filter the ‘cloud-killer’. If you are interested, you can actually buy a very similar filter from Semrock off-the-shelf here!
The plot below shows our ground-based transit, which agrees well with the transits observed by K2 further suggesting that our planet candidate is indeed a planet. Further, these observations allow us to pinpoint the timing of the transit extremely well, allowing us to predict midpoints at the start of the JWST era (~2021-2023) with less than 2minutes of error. Such small errors are important to enable easy scheduling for in-transit spectroscopic follow-up in the future.
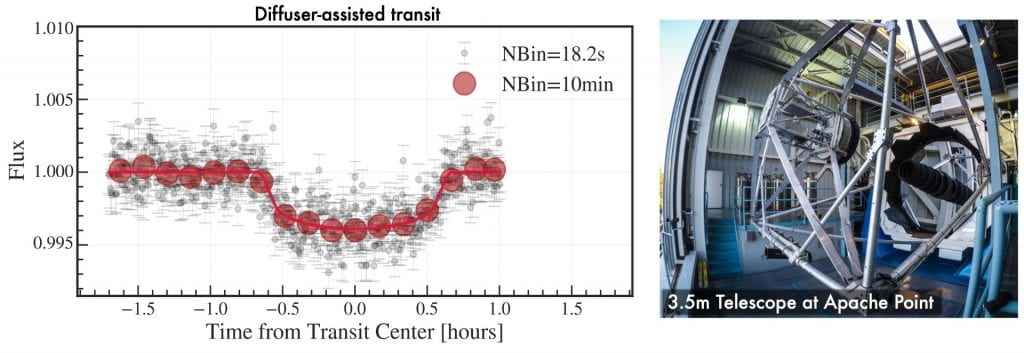
Figure 4: Transit observations of G 9-40b. Left: Ground-based transit measurements—showing the flux of the star as a function of time, showing a clear transit dip—obtained using the ARCTIC imager and the Engineered Diffuser on the 3.5m Telescope at Apache Point Observatory (right). These observations allow us to further characterize key parameters of the planet, including its period and its radius. Image credit: Figure 7e from the paper (left), Gudmundur Stefansson (right).
But what is the diffuser-assisted photometry? Excellent question. This means that we used recent technology called Engineered Diffusers—developed by RPC Photonics—to help conduct the observations. Engineered Diffusers are nano-fabricated devices that you can easily place in a telescope beam-path to mold the focal plane image of a star to a stabilized top-hat shape (Figure 5). In doing so allows you to keep the image of the star stable throughout the night, minimizing photometric errors due to the atmosphere. Further, in spreading out the light over many pixels opens up the possibility to observe bright targets on large telescopes—especially useful in observing G 9-40—with the 3.5m Telescope at Apache Point Observatory. If you are interested in reading more about Engineered Diffusers for astronomy you can read more about them in a past press release here or here, or in our previous paper here.
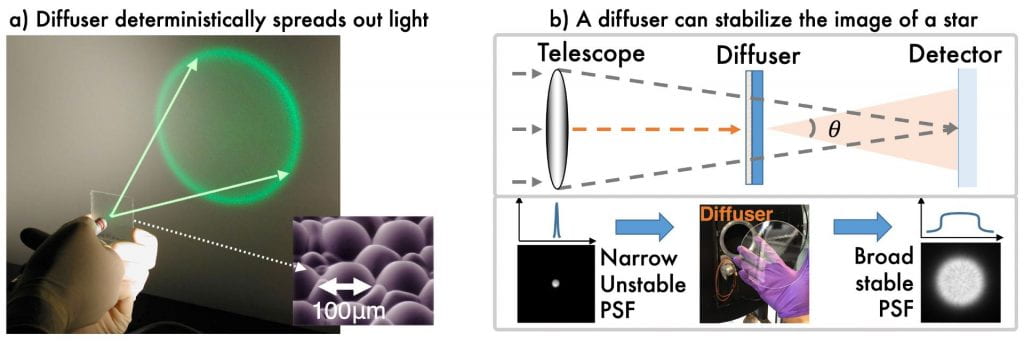
Figure 5: An Engineered Diffuser is capable of molding the image of a star into a broad and stable top-hat shape. b) We used an Engineered Diffuser in the telescope beam path of the 3.5m Telescope at Apache Point Observatory to stabilize the image of the star and allow us to obtain extremely high photometric precisions allowing us to precisely characterize the parameters of G 9-40b. Image credit: RPC Photonics (left), Gudmundur Stefansson (right).
Precision Radial Velocities from HPF
Last but not least, this paper includes some of the first precision near-infrared (NIR) radial velocities (RVs) from the Habitable-zone Planet Finder. In a previous paper led by AJ Metcalf, we showed that HPF is capable of 1.5m/s RV precision in the NIR (see blog post here), enabled by the precise near-infrared HPF Laser Frequency Comb (LFC) also discussed in detail in our previous blog post here. Using precision NIR RVs from HPF enabled by the HPF LFC, we placed an upper limit on the mass of G 9-40b of 12 MEarth. We hope to obtain a robust mass measurement with future RV measurements with HPF in the future. In doing so will allow us to constrain the composition of the planet, and to inform future atmospheric follow-up efforts with JWST.
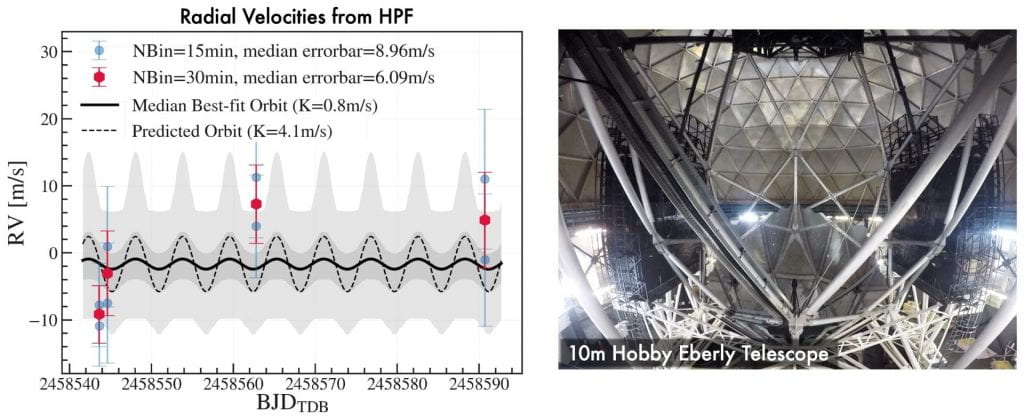
Figure 6: Precise radial velocities from HPF (left) on the 10m Hobby-Eberly Telescope (right) allowed us to place an upper limit on the mass of the planet of 12 Earth masses. We hope to get a further precise mass constraint by continuing to observe G 9-40 in the future. Image credit: Figure 11a from the paper (left), Gudmundur Stefansson (right).