Introduction
One of the signature targets of HPF’s search for nearby exoplanets is Barnard’s star, the second-closest star system to our own Solar system. Barnard’s star made the news in late 2018, when the CARMENES team announced the discovery of a 3.3 Earth mass planet candidate orbiting Barnard’s star on a 233 day orbit. The star has long been a favorite among science fiction writers for setting their stories, so the discovery was met with great excitement by the public as well as the astronomical community. At the same time, the HPF team was observing this star as part of the instrument commissioning campaign, and we began analyzing the data in light of the new announcement. However, the more we looked, and the more data we collected, we did not see evidence for the planet signal. With the addition of nearly 120 new observations from HPF, and an analysis of archival RVs, we have concluded the proposed planet candidate is a false positive. The signal associated with the planet is in reality connected to the star’s 145 day rotation period. This result is the subject of a new research paper led by HPF team member Jack Lubin; it will be published in the Astronomical Journal later this year, and you may access the preprint on arXiv for all of the technical details.
Background
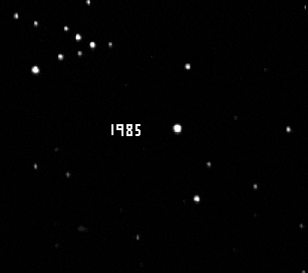
Barnard’s Star can be seen moving relative to background stars in this time-lapse image. Barnard’s Star has the highest on-sky velocity of any star. (Image courtesy Steve Quirk).
Since its discovery by namesake E.E. Barnard in 1916 from the University of California’s Lick Observatory, Barnard’s star has become one of the most well-studied of all the stars in the night sky. Astronomers in all subfields of stellar astrophysics have became fascinated by this star because it has the fastest movement across the sky of any star, relative to background stars. Although, even if Barnard’s star were visible to the naked eye, you wouldn’t notice its motion by eye in your lifetime. We now know that it is the second nearest star system to our own Solar System at just over 5 light years, only beat out by the Alpha Centauri triple star system (thus Barnard’s star is the closest single star).
Barnard’s star has a colorful history when it comes to claims of exoplanets. In 1963, Peter van de Kamp at Swarthmore College published a paper on the star, claiming the first ever detection of an exoplanet. He used a method called astrometry, which measures if a star “wobbles” along the plane of the sky. If the star moves around in an ellipse in a characteristic and periodic motion, then we infer this motion is due to a planet gravitationally tugging on the star. Later that same year he published a follow up paper where he announced a second planet in the system and he refined both masses and orbits.
In 1973, John Hershey, also at Swarthmore College, revisited the same photometric plates as van de Kamp. He determined that all of the stars in the field of view near Barnard’s star were wobbling in the exact same fashion, implying an instrumental error. Hershey was able to point to various upgrades of the telescope that coincided with van de Kamp’s “wobbles”. Also in 1973, George Gatewood from University of Pittsburgh and Heinrich Eichhorn at Wesleyan University published a paper finding no wobble in the star (and therefore no planets) using two different observatories.
Despite these papers showing the two signals van de Kamp published as planets were artifacts of his instrument rather than of astrophysical origin, van de Kamp never conceded. He even published two more papers in 1975 and 1982 refining the orbits and masses of his two planets. Sadly, Peter van de Kamp died in early 1995 believing he had discovered the first exoplanets, just months before the first confirmed exoplanet, 51 Pegasi b, was discovered by Michel Mayor and Didier Queloz, who both shared the 2019 Nobel Prize in Physics for their work. Later publications have definitively ruled out any planets in the Barnard’s star system with the masses and periods of those published by van de Kamp.
More Recent Planet-Hunting Efforts
Recently, an international collaboration led by the CARMENES Spectrometer Instrument team announced the discovery of a 3.3 Earth mass planet candidate orbiting Barnard’s star on a 233 day orbit. They used an expanded RV data set with 700+ observations taken over 23 years using 7 instruments. However, this purported planet’s orbital period is cause for concern. The stellar rotation period of Barnard’s star is around 145 days. How are these two numbers, 233 and 145, related to each other? They are one year aliases of each other.

Examples of signal aliasing: two real signals (gold) are sampled at discrete points, shown by black dots. Using only the measurements at the sampling points, it is impossible to distinguish between the true signal and the higher-frequency alias shown in blue. Image credit: Andrew Jarvis, Wikimedia Commons
Aliasing is a phenomenon in signal processing whereby, due to incomplete sampling of a signal, we can be fooled into thinking a signal has a different period than it actually does . Aliasing is worsened when sampling a signal in unevenly spaced time intervals, as is the unavoidable case for all astronomical observations. Recall, in RV observations we are trying to measure the back and forth movement of the star by the shifting spectral lines. But other phenomena can also produce shifts in the lines, introducing additional signals into our data. Features on the surface of the star itself, such as starspots and other stellar activity, can induce shifts at timescales similar to the the rotation period or its harmonics.
These stellar activity induced signals can mimic a planetary signal, causing a false positive detection. Furthermore, unlike planet signals, stellar activity signals have a decaying nature, which makes aliasing worse. In exoplanet science, aliases most commonly occur with respect to astronomically significant time spans: 1 day, 1 lunar cycle, and 1 year.
New Data, New Conclusion
The HPF team began observing Barnard’s star 3 years ago as part of instrument commissioning. At the time we started, Barnard’s star was not known to host a planet. Soon after, the CARMENES team published their paper detailing the discovery of a planet candidate orbiting Barnard’s star. The HPF team continued observing the star, but as we compiled more and more data, we still saw no evidence for the planet. This prompted us to revisit the data upon which the discovery was based, and perform a joint analysis with our new HPF data.
First, we tested three different models of the system in an effort to discern which one described the data the best:
- A single planet with the same characteristics as claimed in the discovery paper, with no stellar activity
- The same planet, with an additional signal to describe the stellar activity of the star
- No planet, but with stellar activity
In accordance with Occam’s razor, if two models describe the data equally well, then the simpler model is preferred. When we compare our three models using only the data from the paper which claims the planet, we find the model which only accounts for stellar activity fits the data the best. This model is not the simplest model, but its extra complexity is justified by how much better it fits the data. When we repeat the experiment and include the newest HPF data, we find a similar result: our preferred model of the data is one which accounts for the stellar activity of the star, but without the proposed planet.
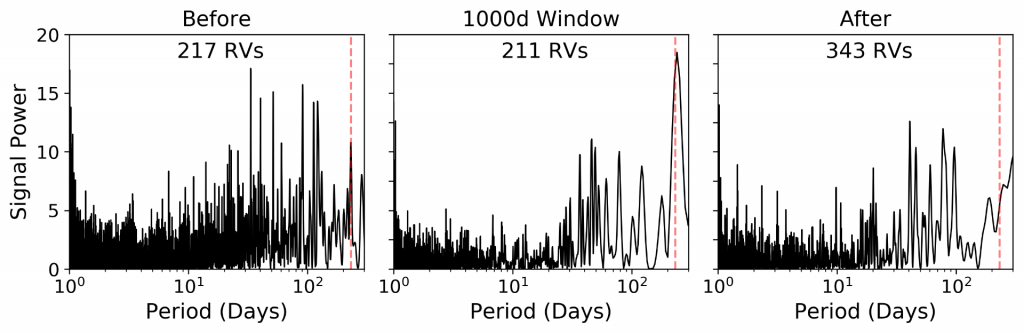
Power spectra of the radial velocities used to detect an exoplanet candidate orbiting Barnard’s star, separated into groups. The period of the purported planet is shown as a dashed red line. The signal appears strongly in a 1000-day region near the middle of the total time series, but not elsewhere.
Next, we reviewed the data year by year, finding that for three consecutive years near the middle of the data set, the signal associated with the 233 day planet was strongest. Meanwhile in other years, especially more recent years, the signal was very weak (even non-existent, like with the HPF data). This was highly concerning because while planet signals are persistent, activity signals are transient. The smoking gun is that in the same years that the signal at 233 days is strongest in the RV time series, there is also a strong signal at this same period in the spectral absorption features sensitive to stellar magnetic activity.
These tests combine to point us towards the conclusion that the proposed planet is instead an artifact of stellar activity manifesting at the one year alias of the rotation period. We are therefore compelled to return the planet of Barnard’s star to the shelf.
Importance and Impact
Barnard’s star is often considered to be the Doppler standard star for RV measurements of M dwarfs. This is due to its high apparent brightness, so even small telescopes can observe it, and its equatorial location, meaning telescopes in both the northern and southern hemispheres can access it. When commissioning new instruments, we often use Barnard’s star as a standard star, among others, because we believe we understand the star well enough that we can reasonably expect to know what the data should look like. Therefore if a new instrument returns different data, we are more likely to attribute the difference to the instrument, not the star. This helps the engineers and scientists refine the instrument and analysis methods.
But Barnard’s star has also been chosen as a Doppler standard because it is thought to be very quiet in nature, with little stellar activity. For decades this has proven true for instruments only capable of measuring signals of a few meters per second and larger. We are finding now, with the newest generation of spectrographs like HPF, that even Barnard’s star is in fact noisy below new, smaller thresholds.
Furthermore, this new paper represents one of the first studies to investigate how stellar activity from a long rotation period plays out over many seasons of data across multiple instruments. The rotation period of Barnard’s star is about 145 days, among the longest known rotation periods of any star! For example, our Sun rotates about once every 25 days, and many stars rotate faster than this. But this long rotation period is a significant portion of an entire observing season for Barnard’s star, which, depending on your latitude, is around 270 days. This long rotation period, coupled with the fact that starspots on M dwarfs can live through 10+ rotations of the star (whereas on our Sun, a starspot might live through only 3 rotations), means that spot-dominated activity on Barnard’s star could maintain high signal power for thousands of days, across multiple observing seasons. This is worrisome when it comes to signal sampling and aliasing. Future exoplanet studies where the host star is an M dwarf with a long rotation period will need to take extra caution when accounting for stellar activity.
In all, Barnard’s star has fascinated astronomers in all subfields for over 100 years, and the exoplanet subfield has a rich history with the star. It is vital to the astronomy community both for its astrophysical insights and for its use as a standard star among Doppler instruments. We may be ruling out this particular planet as a false positive, but the exoplanet community is not done with this star just yet. It is listed on the survey target lists for two new high-precision spectrographs, ESPRESSO and NEID, and the HPF team will continue to monitor Barnard’s star.